Research Spotlight: Exploring Beyond the Hills of Higgs Potential Slope
By Professor Philip Chang
We have learned that like-charges repel, opposite-charges attract. Then, how are so many like-charged protons confined in tiny nuclei? The answer is that they are held together closely by stronger forces that act between tiny subnuclear particles. When this pent-up energy is released, it can create a nuclear explosion, as depicted in the movie Oppenheimer.
The balancing act between repelling electromagnetic forces of protons and the binding nuclear forces is so intricate that the numbers of protons and neutrons have to be just right for a nucleus to be stable; otherwise, a nucleus can split and release the energy. Nature maintains this “account balance” by turning protons into neutrons or vice versa when needed, through the electroweak interaction, which is mediated by the W boson, a heavy elementary particle.
When the magician’s act of turning protons into neutrons was studied in detail, a glaring problem emerged; none of the elementary particles, such as the W boson, could have mass without violating the basic symmetries of quantum field theory! As a remedy, the Higgs boson was introduced. Without the Higgs boson, all elementary particles would be massless, and the creation of atoms, stars, galaxies, and planets would not be possible. Alas, life on Earth would not exist.
According to the theory, Higgs bosons like to self-interact with each other, such that the most preferred natural state of the universe in a vacuum is to be filled with Higgs bosons like molasses rather than nothing. Heavier particles, such as W boson, interact with the sea of Higgs bosons (more precisely, the Higgs field) and feel the molasses of the Higgs boson, while massless particles do not interact, and freely pass through the Higgs molasses.
The expectation of a vacuum filled with Higgs bosons is determined by the shape of the Higgs potential. The self-interaction of the Higgs boson is just right, such that Nature prefers the Higgs field to have a non-zero value at each point in space. Perhaps the universe started with a zero value of the Higgs field, but as time went on, the universe rolled down the Higgs potential hill to a valley of minimal potential energy where a non-zero value is preferred.
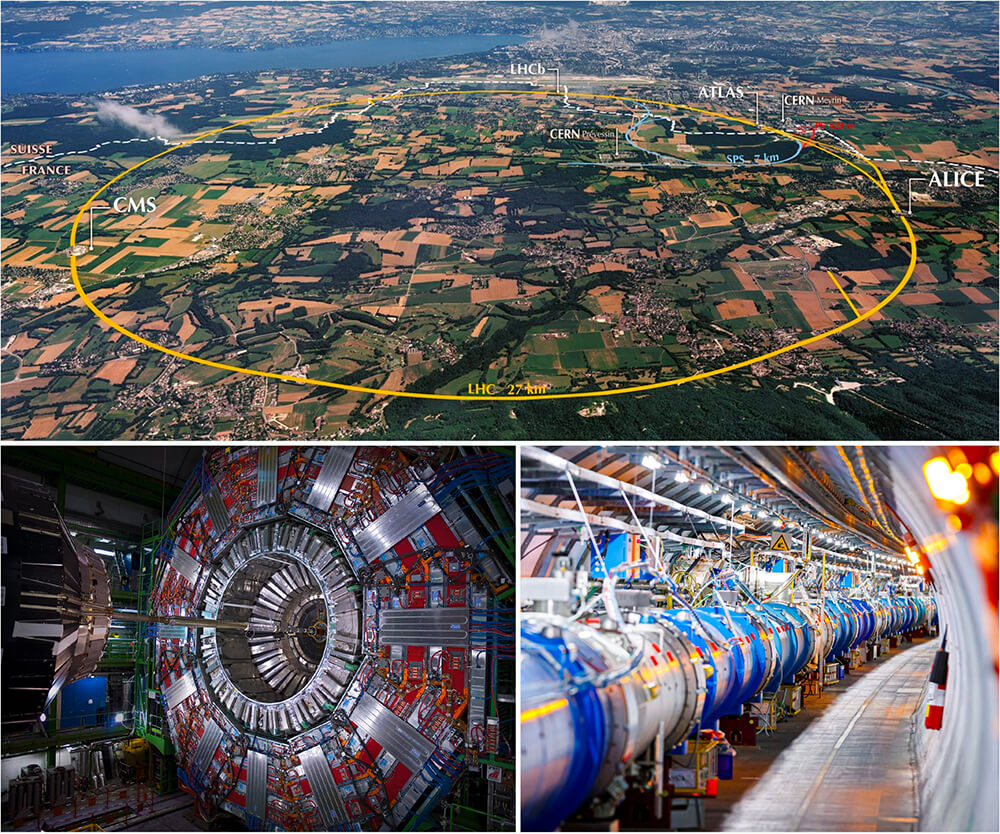
Image credit: CERN
To confirm this theory, it was pertinent to excite the Higgs field, and produce the Higgs boson in the laboratory. In 2012, the Higgs boson was finally discovered at CERN (European Organization for Nuclear Research), leading to the award of a Nobel prize to Peter Higgs and François Englert. The discovery confirmed that there is indeed a Higgs field and that the vacuum is filled with the Higgs field, which provides masses to elementary particles. But how did Nature get here? How did the universe roll into the valley of the Higgs potential?
To answer these questions, one must excite the vacuum once again via the collision of high-energy protons. The vacuum response should provide information about the shape of the Higgs potential. If the Higgs potential is not what we think it is, the experiment may find a deviation from our expectations.
Our lab recently received a grant from the Department of Energy (DOE) to study processes in which multiple Higgs and W bosons are produced via high-energy proton collisions. The main goal of this project is to test whether there are possible deviations in the Higgs potential shape. We specifically search for collision events that produce more than two Higgs or W bosons. These are extremely rare events, but their rate may be enhanced significantly if the Higgs’ potential shape is different from our expectation. If we discover such events and measure them to occur at a rate significantly higher than expected, it would be a sign of new phenomena and would be a greater discovery than the Higgs boson.
We are incorporating artificial-intelligence (AI) techniques and advanced computing methods to improve the science discovery reach. We host the Tier-2 Computing Center (UF Tier-2) at the University of Florida Research Computing, and our lab also incorporates graph neural network (GNN) methods (widely adopted in social media recommendations from AI) in designing better particle-tracking algorithms. We are developing a solution to accelerate and improve the identification of interesting many-boson events using GNN using cutting-edge NVIDIA GPUs, which are part of the University of Florida Tier-2 Center’s computing resources.
The discovery of the Higgs boson has been a giant step towards a better understanding of our universe and its location in the Higgs potential. Now, we must go beyond and explore the hills of the Higgs potential slope.
Return to the Fall 2023 newsletter.